What Is CRISPR Currently Being Used For?
- Published15 Jul 2019
- Author Michael W. Richardson
- Source BrainFacts/SfN
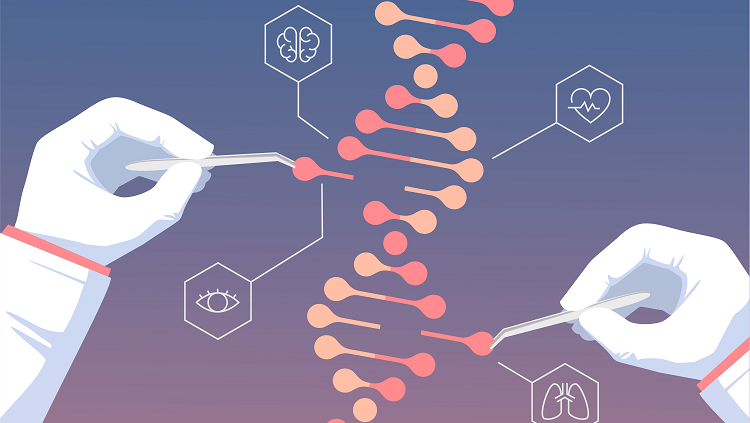
Humans have been tinkering with genetics for thousands of years, even when we didn’t know it. We created modern corgis and pomeranians by breeding pairs of wild wolves for docility, unknowingly selecting the genes controlling for size and temperament. Bananas became a breakfast staple as we continually bred its unappetizing ancestors to produce fruit with the least inedible seeds. It took centuries, but the results speak for themselves.
In the modern day, we manipulate individual genes in organisms, transforming a process taking generations into something that takes a fraction of the time. Scientists could breed a black mouse with a white mouse, locate and eliminate the genes for white fur in the mouse embryo, and rest assured that the baby mouse would grow black fur. Before the year 2000, researchers employed many different technologies to rewrite the genetics of organisms like this. But they were complex, expensive, and specific to a given plant or animal.
Enter CRISPR-Cas9. This gene-editing technique is based on the natural defense mechanism found in some bacteria. It uses a specific enzyme — Cas9 — to identify and eliminate predetermined genes and DNA sequences. It is a cheaper, more effective, and endlessly adaptable form of gene manipulation, and it seems to work in every model organism. Since its initial discovery around the turn of the millennium, scientists haven’t fully realized the impact this technology may have on the field of genetics.
But how does it work, and what kind of treatments can we expect from CRISPR in the future? We asked Sean Ryder, professor of biochemistry and molecular pharmacology at UMass Medical School about this revolutionary breakthrough in genetics.
Where did the idea for CRISPR come from?
In the 1980s, Japanese scientist Yoshizumi Ishino and his team at Osaka University discovered clusters of DNA in some bacteria that contained strange repeats. It wasn’t known why they repeated or what the clusters did, if anything. In 2001, researchers Francisco Mojica and Ruud Jansen coined a name for these repeating sections — CRISPRs, which stands for Clustered Regularly Interspaced Short Palindromic Repeats. A few years later, several independent groups of researchers noticed that these repeating clusters bracketed DNA that came from viruses that had attacked the bacteria before.
They realized that this bacteria was incorporating segments of DNA from viral invaders into its own genome and using it as an early warning system against attacks by the same virus. The CRISPR segments identify and flag the matching DNA in the viruses. But it’s particular enzymes, including one called Cas9, that serve as weapons against the invaders. Cas9 carries a copy of the CRISPR sequence with it, searching for matching DNA in the viruses. Wherever it finds that matching DNA, Cas9 severs it and breaks it apart. Together, they form the CRISPR-Cas9 system.
CRISPR and Cas9 could have just been a clever defense mechanism for bacteria. But in 2012, researchers Jennifer Doudna, Emmanuelle Charpentier, and a separate team led by Lithuanian biochemist Virginijus Siksnys discovered that Cas9 could be reprogrammed to target, destroy, or replace specific genetic sequences, and not just in bacteria and viruses. That’s when its potential for editing genes really appeared.
How does the technology work?
In bacteria, Cas9 carries crRNA — the genetic information of viruses to identify where to make their cuts. Researchers found that they could feed the enzyme fake genetic information, replacing the naturally occurring CRISPRs with an RNA sequence of their choosing, and send Cas9 to attack any gene they liked.
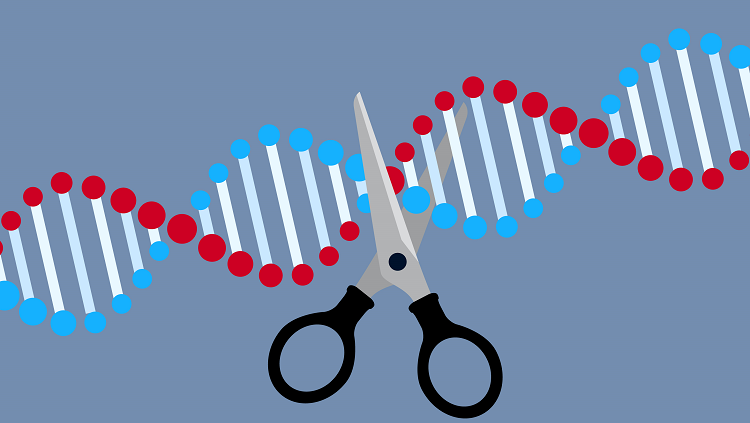
If Cas9 is assigned a specific RNA sequence and delivered to cells, it will hunt down corresponding sequences in the cellular DNA housed in the nuclei and perform a double-strand cut, severing the entire helix at a predetermined point. Severed DNA is an emergency for the cell, so it will immediately try to repair it. The simplest way it does this is by jamming the severed strands back into place and gluing them together. It’s efficient, but it’s also error-prone. And if the strand doesn’t come back together perfectly, it can change the frame of the genetic code, potentially knocking out the gene that has been cut, or rendering it useless. Cas9 can also effectively cut out whole genes by using two guides that cut in two places, removing the intervening sequence, and the strands on either end will be glued together without the cut sequence.
It is possible to use a DNA sequence designed in the lab to repair a gene that has been cut by Cas9. This can be used to repair a broken gene, or to modify its function. However, this technology is less efficient than simply knocking out a gene.
What is CRISPR currently being used for?
In the laboratory, researchers regularly use CRISPR to alter genes in plant, bacteria, and animal models. If you knock out a particular gene in lab mice, you can directly observe what traits or behaviors are affected. This is how a lot of genetic research has been done for decades, but CRISPR makes these studies cheaper, faster, and more reliable.
There are also clinical trials using CRISPR to treat several types of diseases and disorders. For example, at the University of Pennsylvania, researchers are using CRISPR to potentially treat multiple myeloma, a cancer of the blood and bone marrow. They have harvested cells from bone marrow and edited the T cells, part of the immune system, to more accurately target cancer cells before putting them back into the body. The same technique has been used for sarcoma, a similar cancer. We don’t have any results yet, but there is a lot of interest in this approach.
CRISPR-Cas9 is also being used to develop treatments for diseases like sickle cell anemia. In sickle cell anemia, having two bad copies of the Beta-globin causes severe symptoms, while having only one bad copy produces far fewer symptoms. So, researchers have harvested blood cells, run the CRISPR system to repair the one bad copy, and reintroduced the fixed cells back into the bloodstream. These techniques are clever, and they could have amazing ramifications for patients.
Which brain diseases and disorders could CRISPR treat in the future?
CRISPR will probably be most useful for hereditary conditions, such as Huntington’s disease. In Huntington’s, genes from one parent will always cause problems because it is a dominant gene, and there are no reliable treatments currently available. If you can knock out that dominant gene, the body will turn to the recessive, healthy gene for instructions instead. In this case, we can immediately identify an issue from the point of conception and try to act quickly to eliminate that dominant gene.
Hereditary conditions are the most likely targets for gene therapy because CRISPR, while more effective than previous technologies, still doesn’t have a 100 percent success rate. The technology is always more effective in vitro because every cell in your body contains DNA, and a treatment may require the vast majority of those DNA strands to be altered. The fewer cells to edit, the more likely that CRISPR can effectively knock them out. If we know that there is likely to be a genetic problem early on, effective treatment is more likely.
There are still a lot of questions and hurdles that need to be overcome before we can edit entire human genomes. For example, a group of Chinese scientists used CRISPR to edit the genomes of twin girls to have an immunity to a certain strain of HIV. Editing was done during ICSI, intracytoplasmic sperm injection, and in vitro fertilization. Once it was determined that the embryos fertilized in this approach were modified, they were implanted into the mother and carried to term. That made them the first gene-edited children in history. Unfortunately, the work was performed in secret, with little oversight or medical rationale. CRISPR-Cas9 was used to destroy a normal gene in hopes of preventing the possibility of HIV infection. While no data has been published, analyses of information released during the Hong Kong genome editing conference revealed that both children contain at least two different modifications, and it’s not clear whether all cells are modified.
But even if CRISPR-Cas9 worked as planned, we simply don’t know the unintended consequences of altering this gene in humans. We don’t know everything about the human genome. There’s evidence the gene that was edited to prevent this particular strain of HIV also plays a role in intelligence and memory in animals. These girls may have had their cognitive abilities altered in some way. And there’s evidence that the knocked-out gene helped protect against West Nile virus, so they may be more likely to contract that terrible disease in the future. Lastly, and perhaps most disturbing, the modifications could have unintended consequences, as a recent report has shown one particular mutation of CCR5 in humans is deleterious.
I find it extraordinarily risky and dangerous to create mutations in genes we don’t fully understand, without knowing the full consequences of any changes. And when these girls grow up, they could pass on their edited genes. So, it’s not just affecting the patient — it’s affecting their progeny, and their progeny, down the entire family line. In order for me to be comfortable with this kind of gene editing, the technology will have to become more accurate, easier to apply, and capable of precisely editing genes, not just cutting or knocking them out. And I don’t think we’re there yet.
CONTENTPROVIDEDBY
BrainFacts/SfN
References
Heidenreich, M., & Zhang, F. (2015). Applications of CRISPR–Cas systems in neuroscience. Nature Reviews Neuroscience,17(1), 36–44. doi:10.1038/nrn.2015.2
Lander, E. (2016). The Heroes of CRISPR. Cell,164(1-2), 18–28. doi:10.1016/j.cell.2015.12.041